Invertebrates in the medical service of man: Part 3 – The Research Assistants
Charles Savona-Ventura MD DScMed FRCOG AccrCOG MRCPI
Professor of Obstetrics & Gynaecology
Faculty of Medicine & Surgery, University of Malta
The invertebrates have generally been medically classed as disease vectors, though a number of species have been utilized in the management of surgical conditions or for the biotherapeutic properties of their secretions.1,2 A number of species have been essential tools in frontline research studies which have in some instances received international acclaim.
Phylum Mollusca; Class: Cephalopoda;
Several species of Squids, Cuttlefish and Octopi
Sepioteuthis lessoniana; Sepioteuthis sepioidea; Lolliguncula brevis;
Loligo opalescens; Loligo pealei; Illex illecebrosus;
Sepia officinalis; Octopus vulgaris
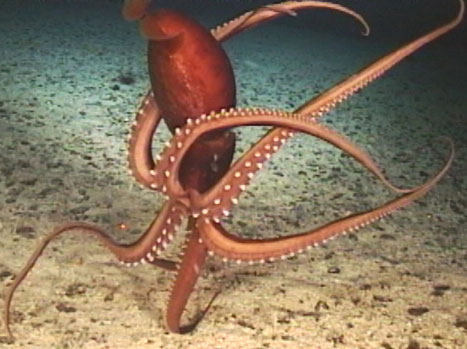
Loligo sp.
In 1909, L.W. Williams noted that the squid possessed a giant nerve cell or more specifically a large projection from the cell which is called an axon. These observations slipped into obscurity until 1936, when the Professor of Anatomy J.Z. Young rediscovered this curiosity. Using a pair of electrodes, Young stimulated surrounding nerve fibers and found that he could only produce large muscle contractions in the mantle when the large vessel-like structure remained intact, confirming that the structure was indeed a single, huge nerve axon. Scientists quickly appreciated the significance of Young’s finding in presenting a working neurological model. This enabled scientists to initiate a number of research projects aimed at understanding neural function.
Based on squid models, the British scientists, Alan Hodgkin and Andrew Huxley, in a series of papers published during the 1950s described the behavior of nerve impulses. This sterling work was in 1963 awarded the Nobel Prize. Further researchers working in the field of neural physiology using the squid model were also in subsequent years made Nobel Laureates. These included the American George Wald, honored in 1967 for his research on chemical and physiological processes in the eye, and the British Bernard Katz, honored in 1970 for his discoveries concerning the role played by chemicals in nerve impulses.
Squid research was hampered by the difficulty in breeding these animals in captivity. Several species of squid were experimented with but problems were encountered either because the species was too small or because it generally lived in cold waters. Finally, in 1987 experimentation with the warm-water species Sepioteuthis lessoniana yielded promising results and presented researchers with a laboratory-bred animal. The cephalopods have been useful in elucidating physiological information on membrane biophysics, the ion channel, neuron development and injury, cell biology, muscle biomechanics, brain physiology, chemical neural reception, vision and oculomotor functions, equilibrium receptor systems, behavior and neural pharmacology, transmitter functions, melanin synthesis and developmental biology.
Phylum: Arthropoda; Class: Merostomata; Order: Xiphosura
Horseshoe Crab – Limulus poluphemus
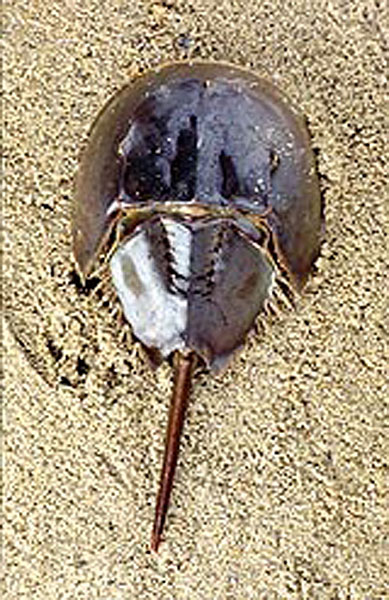
Another invertebrate contributor to the understanding of neural processes was the Horseshoe Crab. Much of what we know about the function of our eyes is the result of studies that began over 50 years ago on the large, compound eyes of the horseshoe crab. Its eyes have a relatively simple construction, and the optic nerve is readily accessible. In addition, it is easy to keep Limulus alive in the laboratory, making it an ideal animal for eye research. The cornea of Limulus eyes was first examined in 1782, while the 19th century was to result in the investigation into the structure of the median eyes (each with a single lens) and the lateral eyes (composed of small hexagonal eyes called ommatidia). Physiological studies on the electrical impulses in the horseshoe crab optic nerve were carried out in 1928 by Dr. H. Keffer Hartline, whereas in 1932, electrical responses were recorded for the first time from a single visual receptor of the Limulus eye. In 1967, Dr. Hartline was awarded the Nobel Prize for his continuing research on horseshoe crab vision. His researches had elucidated how sensory cells in the retina help the brain process visual cues, enabling horseshoe crabs to see lines, shapes, and borders. This mechanism, called lateral inhibition, allows horseshoe crabs to distinguish mates in murky water. Research of this type was helpful for understanding human eye diseases like retinitis pigmentosa, which causes tunnel vision and can lead to total blindness.3 Other researchers have utilized the horseshoe crab’s eyes to identify the visual pigment as rhodopsin (1960), and also related light sensitivity to a circadian clock mechanism in the horseshoe crab’s brain that enhances night vision. Building on the Hartline’s lateral inhibition and circadian clock mechanism research, the American ophthalmologist Dr. Robert Barlow and his colleagues are investigating the role of vision in potential mate selection. By using dedicated computer models, Dr. Barlow has analyzed how the brain of a horseshoe crab processes signals transmitted from the eyes and optic nerve. It is hoped that in the future, decoding this pathway may provide valuable information for correcting human vision disorders. An extract of the horseshoe crab’s blue copper-based blood – lysate – is used to test the purity of a number of medicinal properties. Furthermore certain properties of the shell are used to help speed blood clotting mechanisms and to produce absorbable sutures.
Phylum: Arthropoda; Class: Insecta; Order: Diptera; Family: Drosphilidae
Fruit Fly – Drosophila melanogaster [Maltese – Ferminalle]
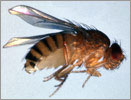
The fruit fly Drosophila has long been a major contributor to our fundamental understanding of genetic processes serving as the primary organism in the development of the chromosome theory of heredity. As with most of the long-established model organisms, the initial choice for using this species was for practical reasons. The fruit fly is small and has a simple diet and hence large numbers of flies can be maintained inexpensively in the laboratory. The life cycle is also very short, taking about two weeks, so large-scale crosses can be set up and followed through several generations in a matter of months. Fruit flies also have large polytene chromosomes, whose barcode patterns of light and dark bands allow genes to be mapped accurately.
In the early 1900s, American-based geneticists began to make important contributions to genetic research. Working at Columbia University in 1910, Thomas Hunt Morgan conducted experiments in fruit flies, mainly Drosophila melanogaster, and established that some genetically-determined traits were sex-linked. Morgan’s work was followed up by his students – Calvin Bridges in 1913 established that genes were located on chromosomes; while Alfred Sturtevant in 1913 determined that the genes were arranged on the chromosomes in a linear fashion and further demonstrated that the gene for any specific trait was in a fixed location or locus. Yet another of Morgan’s students, Herman J. Muller, in 1926 discovered methods for artificially producing mutants in fruit flies by ionizing radiation and other mutagens, thus discovering the origin of new genes by mutations. These studies opened a new vista for understanding the principles of genetics, mutations and chromosome behavior. A second major contribution of Drosophila research has been in our understanding of development. The development of a single egg cell into a many-celled organism is a complex process. The system of patterning genes and signaling molecules in the fruit fly leads to the direct development of different organs in their proper locations. In 1980, Christiane Nusselein-Volhard and Eric Wieschaus performed the first genome-wide mutational screen in an attempt to identify all genes involved in development. In acknowledgement for the work on Drosophila developmental genetics, Ed Lewis, Christiane Nusslein-Volhard and Eric Wieschaus were awarded the Nobel Prize for medicine and physiology in 1995.4
The fly genome was sequenced in the year 2001 by J. Craig Venter and the Celera corporation, although much of the work had already been laid by the Berkelely, European, and Canadian Drosophila genome projects. The genome is made up of 165 million base pairs in length (spread over four chromosomes) and contains approximately 14,000 genes. The full sequence of the Drosophila genome adds to the usefulness of the fly as a model organism. Now that the Drosophila genome has been sequenced, the genetic code will greatly facilitate positional cloning methods. The new sequence has also revealed previously unknown counterparts to human diseases, most notably cancer and neurological diseases. With this new knowledge, it is possible to model human diseases and disease pathways in flies. Additionally, it is now possible to systematically study entire networks of genes at once, rather than individual pathways. Consequently, studies in fruit flies will reveal important insights into human physiology and medicine. Finally, the fruit fly could be the key to answering the great question of how a genome can give rise to an organism without any instructions for doing so, possibly serving as the key for artificial organ production from stem cells.
References
- Savona-Ventura C. Invertebrates in the medical service of man: Part 1 – The Biotherapeutic Worms. The Synapse Magazine 2007; 1:14, 18.
- Savona-Ventura C. Invertebrates in the medical service of man: Part 2 – The Insect Surgeons. The Synapse Magazine 2007; 2:16-7.
- Hartline HK, Wagner HG, Ratcliff F. Inhibition in the Eye of Limulus. Journal of General Physiology 1956; 39(5):651-73.
- Rubin GM and Lewis EB. A Brief History of Drosophila’s Contributions to Genome Research. Science 2000; 287:2216-8.